Camelids, including camels, llamas, and alpacas, possess a unique antibody system that has been gaining significant attention in recent years. These animals produce not only conventional antibodies but also a distinct class of antibodies known as heavy-chain antibodies (HCAbs), which lack light chains. The discovery of HCAbs led to the development of a new type of antibody fragments - single-domain antibodies or nanobodies. The properties of HCAbs and nanobodies have been very promising in the field of medicine and diagnostics, so the experiments around them continue.
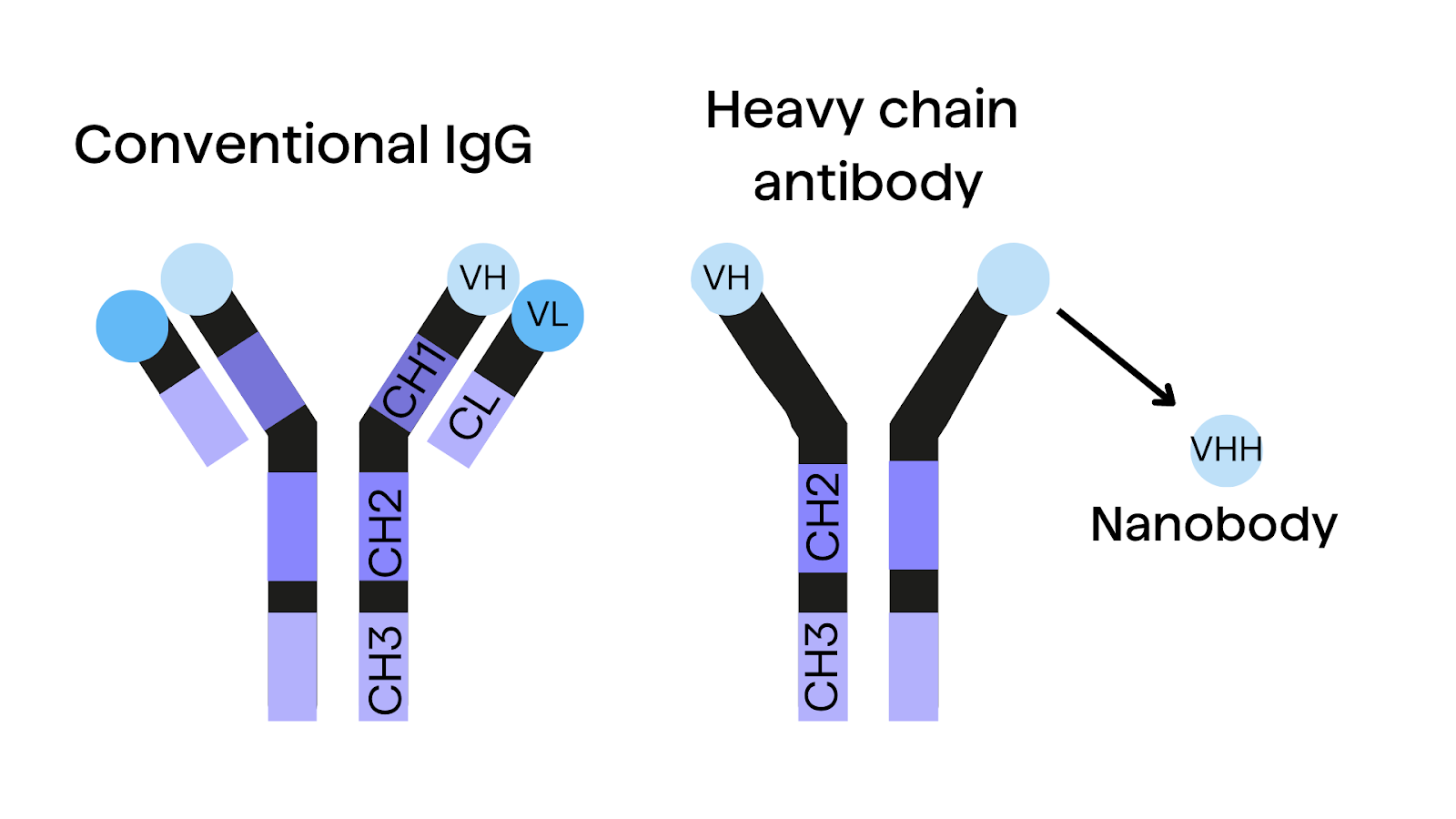
Evolutionary Origins and Advantages of Camelid antibodies
Camelids belong to the order Cetartiodactyla and share an evolutionary distance of approximately 92 million years from humans, comparable to the divergence between humans and mice5,6. This evolutionary distance has contributed to the development of unique antibody structures in camelids, such as HCAbs and nanobodies.
Nanobodies offer numerous advantages for laboratory and therapeutic applications. They are small (approximately 15 kDa ) in comparison to conventional IgGs (around 150 kDa)2,25, stable under a wide range of temperature and chemical conditions2, easy to clone2, and amenable to screening using phage display7. Additionally, camelid VHHs can recognize distinct epitopes that are inaccessible to human antibodies, as demonstrated in the case of SARS-CoV-2 nanobodies9. Their small size also simplifies humanization, manufacturing, and tissue penetration, although it can lead to rapid clearance and poor pharmacokinetic properties, which can be addressed by engineering them into conjugated or multivalent formats9.

Structural and Functional Adaptations of HCAbs
Some studies show differences between VH and VHH in camelid repertoires. In a comparative analysis from Li et al3 of the immune repertoire between VH and VHH in three Bactrian camels, HCAbs seems to exhibit longer CDR3 lengths, with an average of 18 amino acids compared to 13 amino acids in conventional antibodies3. This increase in CDR3 length again may enable access to sterically hindered sites, such as ligand-binding pockets and enzyme active sites3.
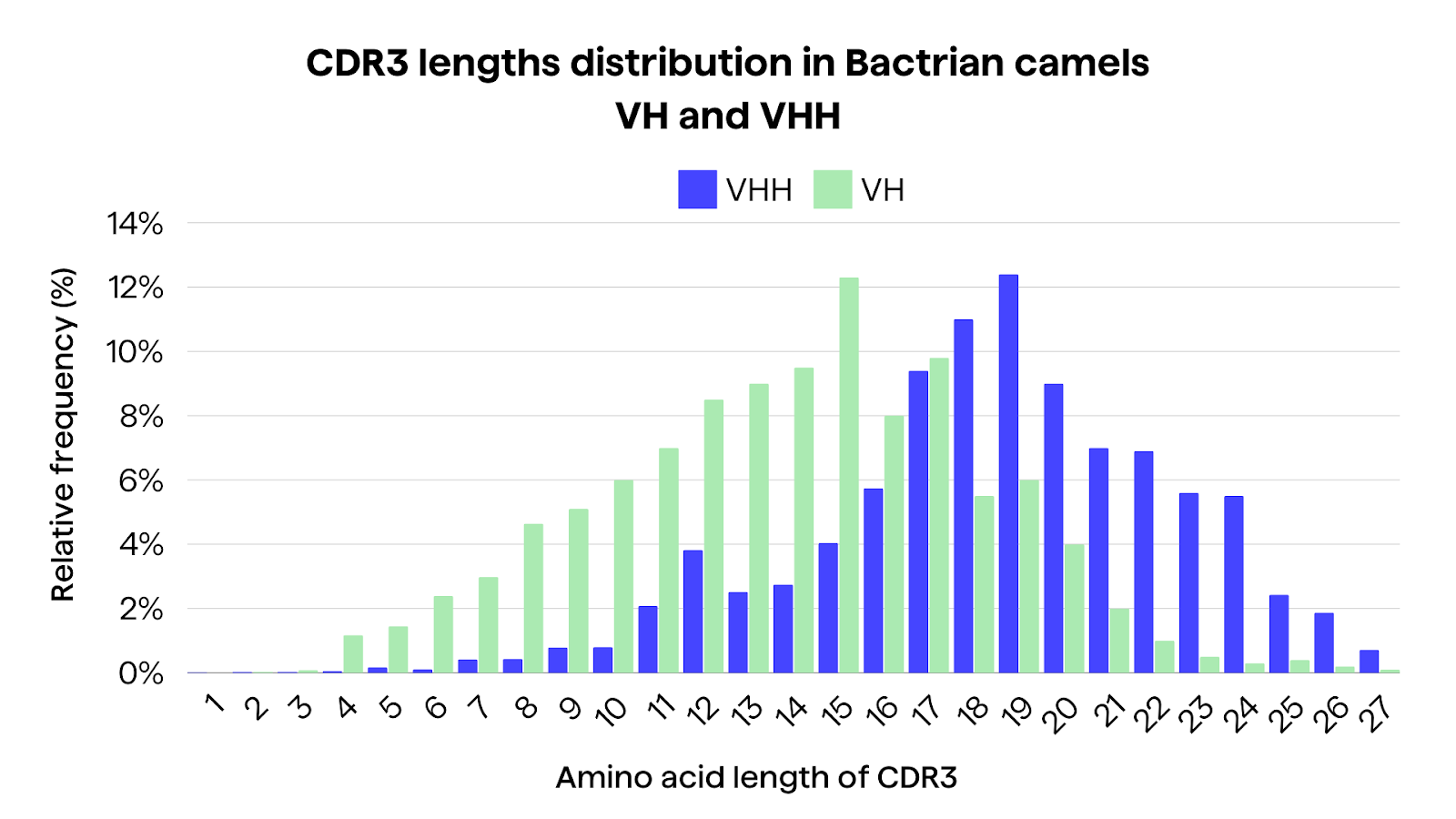
Additionally, HCAbs demonstrated a higher mutation rate (12.3% vs. 8.3% in conventional antibodies) and characteristic amino acid substitutions, particularly at positions 49, 50, 52, 54, 57, 96, and 1013. These substitutions may likely contribute to the increased solubility and structural stability of HCAbs, compensating for the lack of a light chain3.
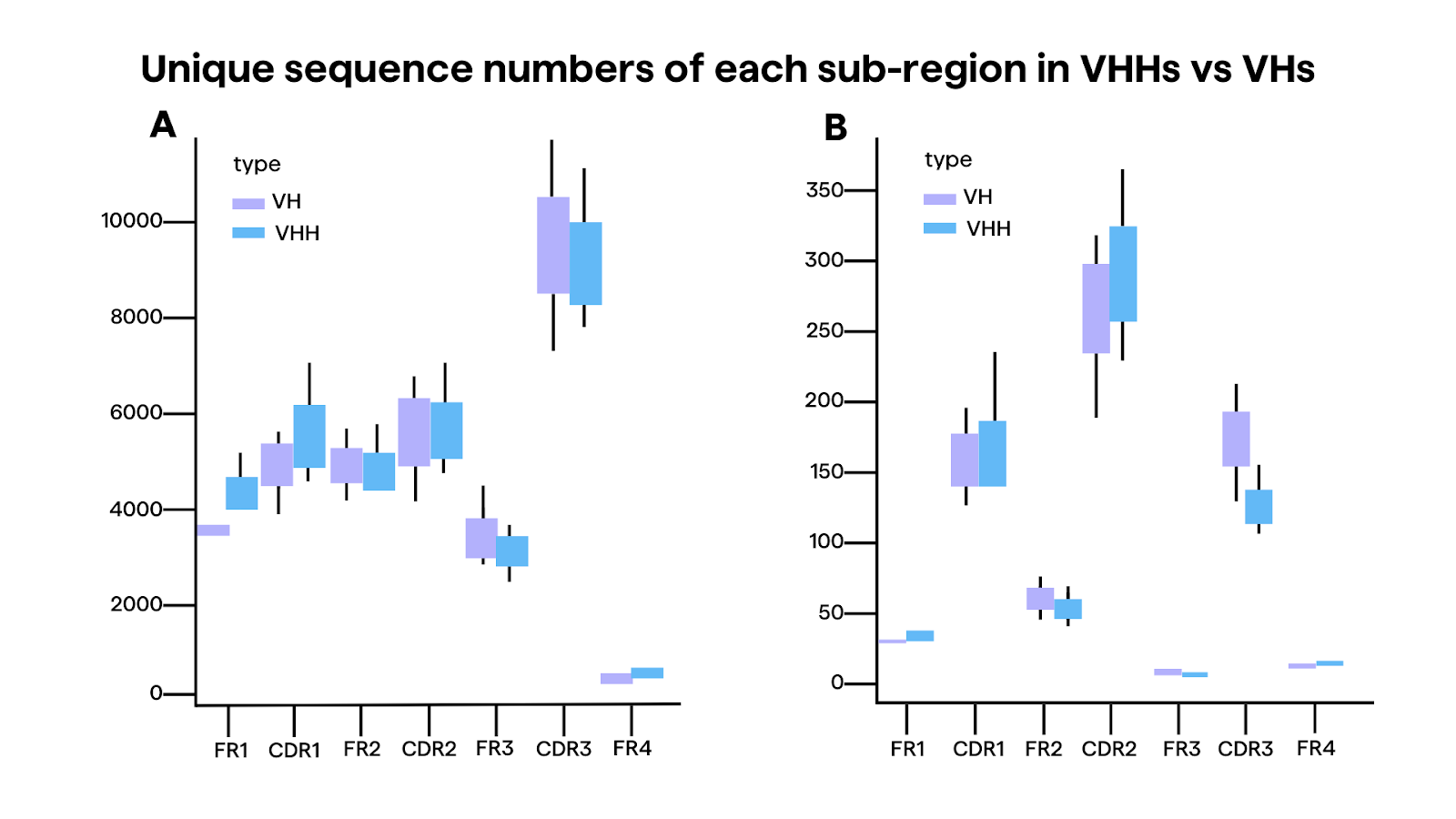
Furthermore, the distribution of cysteine codons, which form disulfide bonds to stabilize the antibody structure, seemed significantly higher in VHHs compared to VHs, especially in the CDR1 region (9.8% vs. 0.5%)3. This suggests that HCAbs may have evolved additional disulfide bonds to maintain structural integrity in the absence of a light chain.
Structural studies have revealed that the CDRs of camelid sdAbs can adopt a wide range of conformations, contributing to their ability to recognize diverse epitopes. Examples include intracellular targets10,11 or epitopes concealed from mAbs in protein structures12, G protein-coupled receptors13,14, and ion channels15. For instance, the CDR3 loop can form extended or protruding structures that can access clefts or cavities on antigens, while the CDR1 loop can participate in antigen binding more extensively than in conventional antibodies. Additionally, the CDR2 loop often plays a crucial role in shaping the conformation of the CDR3 loop and determining antigen specificity.5
Non-Classical VHH Clones and Genomic Organization
While genomic and cDNA studies have shed light on the camelid VHH gene repertoire, a complete picture of the full germline V gene repertoires for both heavy and light chains is still lacking17. Preliminary investigations suggest that camelid VHH genes are highly homologous to the human VH3 family, with some key amino acid substitutions in the FR2.18 However, recent studies have also identified novel camelid V genes closely related to human IGHV5 and IGHV7 families.19 Ongoing efforts to fully characterize the camelid germline V gene repertoires will further our understanding of the evolutionary origins and diversification mechanisms of HCAbs.5
A subset of "non-classical" VHH clones, comprising approximately 8% of the total, are derived from conventional VH genes rather than the typical VHH genes16,17. These non-classical VHHs exhibit distinct features, such as a higher frequency of arginine substitution at the first position of the framework 4 region and shorter CDR3 lengths compared to classical VHHs3.
The genomic organization of the camelid IgH locus sheds light on the origins of conventional and homodimeric IgGs4. The IgH locus contains an intermixed arrangement of VHH genes, which encode the variable regions of homodimeric IgGs, and conventional VH genes, which encode the variable regions of tetrameric IgGs4. Downstream of the V gene cluster is a single DH-JH gene cluster, followed by the C gene region, which includes both CHH genes encoding the constant regions of homodimeric IgGs and conventional CH genes4.

Phylogenetic analysis suggests that the VHH genes emerged from pre-existing VH genes of the IGHV3 subgroup, while the IGHV1 and IGHV2 subgroups contain only conventional VH genes.26 This supports a model in which homodimeric IgG-expressing B cells develop through an IgM+ stage, similar to the development of conventional IgG-expressing B cells26,27.
Therapeutic and Diagnostic Applications
The unique properties of camelid antibodies, particularly nanobodies, have opened up a wealth of opportunities for therapeutic and diagnostic applications. As of 2020, there were at least 37 camelid antibodies in clinical trials and numerous others in preclinical stages of development2.
Caplacizumab21, a bivalent nanobody was the first approved monoclonal antibody obtained from a non-rodent animal source2. It targets von Willebrand factor for the treatment of thrombotic thrombocytopenic purpura and thrombosis.22
Nanobodies have shown promise in targeting challenging and conserved drug targets, such as enzymes and G-protein-coupled receptors (GPCRs)20. Their long CDR3 regions enable them to access sterically hindered sites, making them attractive candidates for developing inhibitory, antagonist, and agonist antibodies against these targets2.
Furthermore, the ability of camelid antibodies to recognize distinct epitopes from human antibodies has been harnessed in the development of SARS-CoV-2 nanobodies that can bind to regions of the virus inaccessible to human MAbs23. This highlights the potential of camelid antibodies in addressing emerging infectious diseases and other challenging targets.
Future Directions and Concluding Remarks
The camelid antibody repertoire represents a valuable resource for antibody discovery and development, offering unique structural and functional properties that complement conventional antibody platforms. While camelids provide attractive features for antibody discovery, practical considerations such as the animal’s large size, limited accessible B cell repertoire, and mammalian genome homology to humans also present challenges2.
Additionally, the increasing availability of camelid genomic and transcriptomic data, coupled with advanced computational tools, will facilitate a deeper understanding of the evolutionary origins, diversification mechanisms, and structural adaptations of camelid antibodies3,4.
While camelid sdAbs offer several advantages over conventional mAbs and antibody fragments, their novel nature also presents unique regulatory challenges5. As the first therapeutic products of their kind, sdAb-based therapeutics will likely face increased scrutiny from regulatory agencies regarding their safety, immunogenicity, and manufacturing processes. Additionally, establishing standardized analytical and characterization methods for these single-domain antibodies may pose challenges, as they differ structurally from conventional mAbs. Overcoming these regulatory hurdles will be crucial for the successful development and approval of sdAb-based therapeutics5.